Virgo interferometer
The Virgo interferometer is a large Michelson interferometer designed to detect gravitational waves predicted by the general theory of relativity. It is located in Santo Stefano a Macerata, near the city of Pisa, Italy. The instrument's two arms are three kilometres long, hosting its mirrors and instrumentation inside an ultra-high vacuum.
![]() | |
![]() Country with institutions contributing to EGO and the Virgo Collaboration Country with institutions contributing to the Virgo Collaboration | |
Formation | 1993 |
---|---|
Type | International scientific collaboration |
Purpose | Gravitational wave detection |
Headquarters | European Gravitational Observatory |
Location | |
Coordinates | 43.6313°N 10.5045°E |
Region | Italy |
Fields | Basic research |
Spokesperson | Gianluca Gemme |
Affiliations | LVK (LIGO Scientific Collaboration, Virgo Collaboration and KAGRA collaboration) |
Budget | About ten million euros per year |
Staff | Around 850 people participate in the Virgo Collaboration |
Website | www |
Virgo is hosted by the European Gravitational Observatory (EGO), a consortium founded by the French CNRS and Italian INFN.[1] The Virgo Collaboration operates the detector and is composed of more than 700 members, representing 129 institutions in 16 different countries.[2] Other interferometers similar to Virgo have the same goal of detecting gravitational waves, including the two LIGO interferometers in the United States (at the Hanford Site and in Livingston, Louisiana) and the Japanese interferometer KAGRA. Since 2007, Virgo and LIGO have agreed to share and jointly analyze the data recorded by their detectors and to jointly publish their results.[3] Because the interferometric detectors are not directional (they survey the whole sky) and are looking for signals which are weak and infrequent, simultaneous detection of a gravitational wave in multiple instruments is necessary to confirm the signal validity and to deduce the location of its source.
The interferometer is named after the Virgo Cluster, a cluster of about 1,500 galaxies in the Virgo constellation, about 50 million light-years from Earth. Built as part of a European effort to detect the gravitational waves predicted by general relativity, it now plays a significant part in the global gravitational wave detector network, as gravitational wave astronomy takes its first steps. It has participated in a number of detections, and periodically undergoes improvements to enhance its sensitivity and increase its performance.
Organization
The Virgo experiment is managed by the European Gravitational Observatory (EGO) consortium, created in December 2000 by the CNRS and INFN.[4] The Dutch Institute for Nuclear and High-Energy Physics Nikhef later joined as an observer and eventually a full member. The EGO is responsible for the Virgo site, in charge of the construction, maintenance, and operation of the detector, as well as its upgrades. The goal of the EGO is also to promote research and studies about gravitation in Europe.[1]
In addition, the Virgo Collaboration regroups all the researchers working on various aspects of the detector. As of May 2023, around 850 members, representing 142 institutions in 16 different countries, are part of the collaboration.[2][5] This includes institutions from France, Italy, the Netherlands, Poland, Spain, Belgium, Germany, Hungary, Portugal, Greece, Czechia, Denmark, Ireland, Monaco, China, and Japan.[6]
The Virgo Collaboration is also part of the larger LIGO-Virgo-KAGRA (LVK) Collaboration, regrouping scientists from the other major gravitational waves experiment, for the purpose of carrying a joint analysis of the data which is crucial for gravitational wave detections.[7] It first started in 2010 as the LIGO-Virgo Collaboration, and was extended when KAGRA joined in 2021.[8]
History
The Virgo project was approved in 1992 by the French CNRS and in 1993 by the Italian INFN, the two institutes at the origin of the experiment. The construction of the detector started in 1996 in the Cascina site near Pisa, Italy, and was completed in 2003. After several observing runs without detection, the interferometer was shut down in 2011 to allow for significant upgrades as part of the Advanced Virgo project. It started making observations again in 2017, quickly making its first detections along with the LIGO detectors.
Conception
Although the concept of gravitational waves is more than 100 years old, having been predicted by Einstein in 1916,[9] it was not before the 1970s that serious projects for detecting them started to appear. The first were the so-called Weber bars, invented by Joseph Weber,[10] which could in principle detect gravitational waves. This triggered a number of projects, such as AURIGA. While none of these projects succeeded, they did spark the creation of many research groups dedicated to gravitational wave search.[11]
The idea of a large interferometric detector began to gain credibility, and in 1985, the Virgo project was imagined by the Italian researcher Adalberto Giazotto and the French researcher Alain Brillet after they met in Rome. One of the key ideas that set Virgo apart from other projects was targeting low frequencies (around 10 Hz), whereas most projects focused on higher frequencies (around 500 Hz); many believed at the time that it was not doable, and only France and Italy started working on the project,[12] which was first presented in 1987.[13] After being approved by the CNRS in 1992 and the INFN in 1993, construction of the interferometer began in 1996, with the aim of beginning observations by the year 2000.[14]
The first goal of Virgo was to directly observe gravitational waves. The study over three decades of the binary pulsar 1913+16, whose discovery was awarded the 1993 Nobel Prize in Physics, had already led to indirect evidence of the existence of gravitational waves. The observed decrease over time of this binary pulsar's orbital period was in excellent agreement with the hypothesis that the system is losing energy by emitting gravitational waves.[15]
Initial Virgo detector
In the 2000s, the Virgo detector was first built, commissioned, and operated. The instrument reached its design sensitivity to gravitational wave signals. This initial endeavour was used to validate the Virgo technical design choices; it also demonstrated that giant interferometers are promising devices for detecting gravitational waves in a wide frequency band.[16][17] This original detector is generally referred to as the "initial Virgo" or "original Virgo".
The construction of the initial Virgo detector was completed in June 2003[18] and several data-taking periods followed between 2007 and 2011.[19] Some of these runs were done in coincidence with the two LIGO detectors. The initial Virgo detector recorded scientific data from 2007 to 2011 during four science runs.[20] There was a shut-down of a few months in 2010 to allow for a major upgrade of the Virgo suspension system: the original steel suspension wires were replaced by glass fibers in order to reduce the thermal noise.[21]
However, the initial Virgo detector was not sensitive enough to detect such gravitational waves. After several months of data taking with the upgraded suspension system, the initial Virgo detector was shut down in September 2011 to begin the installation of Advanced Virgo.[22]
Advanced Virgo detector
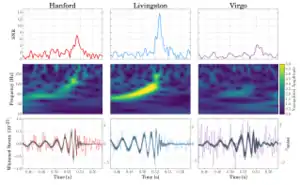
The Advanced Virgo detector aims to increase the sensitivity by a factor of 10, allowing it to probe a volume of the Universe 1,000 times larger, making detections of gravitational waves more likely.[12][23] It benefits from the experience gained with the initial detector and subsequent technological advances.
The Advanced Virgo detector keeps the same vacuum infrastructure as initial Virgo, with four additional cryotraps located at both ends of both three-kilometre-long arms to trap residual particles coming from the mirror towers, but the remainder of the interferometer has been significantly upgraded. The new mirrors are larger (350 mm in diameter, with a weight of 40 kg), and their optical performance has been improved. The critical optical elements used to control the interferometer are under vacuum on suspended benches. A system of adaptive optics was to be installed to correct the mirror aberrations in-situ. In the final Advanced Virgo configuration, the laser power will be 200 W.[24]
Advanced Virgo started the commissioning process in 2016, joining the two advanced LIGO detectors ("aLIGO") for a first "engineering" observing period in May and June 2017.[25] On 14 August 2017, LIGO and Virgo detected a signal, GW170814, which was reported on 27 September 2017. It was the first binary black hole merger detected by both LIGO and Virgo (and the first one for Virgo).[26][27]
Just few days later, GW170817 was detected by the LIGO and Virgo on 17 August 2017. The signal was produced by the last minutes of two neutron stars spiralling closer to each other and finally merging, and is the first gravitational wave observation which has been confirmed by non-gravitational means.[28][29]
After further upgrades, Virgo started the third observation run ("O3") in April 2019, planned to last one year, followed by further upgrades.[30] On the 27th of March 2020, the O3 run was suspended because of the COVID-19 pandemic.[31]
The upgrades currently underway are part of the "Advanced Virgo +" program, divided in two phases, the first one preceding the O4 run and the second one preceding the O5 run. The first phase focuses on the reduction of quantum noise by introducing a more powerful laser, improving the squeezing introduced in O3, and implementing a new technique called signal recycling; seismic sensors will also be installed around the mirrors. The second phase will then try to reduce the mirror thermal noise, by changing the geometry of the laser beam to increase its size on the mirrors (spreading the energy on a larger area and thus reducing the temperature), and by improving the coating of the mirrors; the end mirrors will also be significantly larger, requiring improvements to the suspension. Further improvements to the quantum noise are also expected in the second phase, building upon the changes from the first phase.[32]
Future
The gravitational wave observatories LIGO, Virgo, and KAGRA are coordinating to continue observations after the stop caused by COVID, and plan to start the O4 observing run in May 2023. Virgo projects a sensitivity goal of 80–115 Mpc for binary neutron star mergers (sensitivities: LIGO 160–190 Mpc, KAGRA greater than 1 Mpc). This run is expected to last for 18 months instead of the 12 months initially envisioned, in order to accommodate upgrades planned for the following run.[33] On 11 May 2023, Virgo announced that it will not join at the beginning of O4, as the interferometer is not stable enough to reach the expected sensitivity and needs to undergo the replacement of one of the mirrors, requiring several weeks of work.[34]
Following this run, the detector will once again be shut down to undergo upgrades, including an improvement in the coating of the mirrors. A fifth observing run (O5) is currently planned for the beginning of 2027, with a projected sensitivity of 150–260 Mpc for Virgo (although these plans are only provisional).[33]
No official plans have been announced for the future of the Virgo installations following the O5 period, although projects for further improving the detectors have been suggested.[35]
Science case

The Advanced Virgo interferometer aims to detect and study gravitational waves from astrophysical sources in the Universe. The main known gravitational-wave emitting systems within the sensibility of ground-base interferometers are: black hole and/or neutron star binary mergers, rotating neutron stars, bursts and supernovae explosions, and even the gravitational wave background due to the Big Bang. Moreover, gravitational radiation may also lead to the discovery of unexpected and theoretically predicted exotic objects.
Transient sources
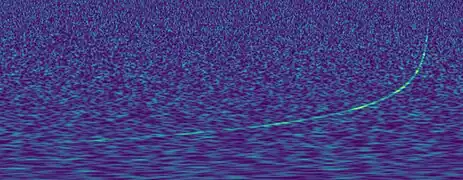
Coalescences of black holes and neutron stars
When two massive and compact objects such as black holes and neutron stars orbit each other in a binary system, they emit gravitational radiation and, therefore, lose energy. Hence, they begin to get closer to each other, increasing the frequency and the amplitude of the gravitational waves; this first phase of the coalescence phenomenon, called the "inspiral", can last for millions of years. As the objects get closer to each other and orbit faster, the amplitude and frequency of the gravitational signal increase, accelerating the process and giving it a characteristic shape known as the "chirp", as it resembles the sound emitted by some birds. This culminates in the merger of the two objects, eventually forming a single compact object (generally a black hole). The part of the waveform corresponding to the merger has the largest amplitude and highest frequency, and can only be modeled by performing numerical relativity simulations of these systems. In the case of black holes, a signal is still emitted during a few seconds after the merger, while the new black hole "settles in"; this signal is known as the "ringdown". The interferometer is only sensitive to the late phase of the coalescence of black hole and neutron star binaries: only between several milliseconds and a second of the whole process can be observed. All detections so far have been of black hole or neutron star mergers.[36][37]
Bursts and supernovae
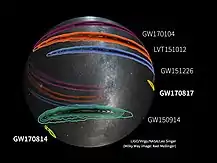
Any signal lasting from a few milliseconds to a few seconds is considered a gravitational wave burst. Supernovae explosions—the gravitational collapse of massive stars at the end of their lives—emit gravitational radiation that can be seen by the Advanced Virgo interferometer.[38] A multi-messenger detection (electromagnetic and gravitational radiation, and neutrinos) would help to better understand the supernovae process and the formation of black holes.[39]
Continuous sources
The main expected sources of continuous gravitational waves are neutron stars, very compact objects resulting from the collapse of massive stars. In particular, pulsars are special cases of neutron stars that emit light pulses periodically: they can spin up to hundreds of times per second (the fastest spinning pulsar currently known is PSR J1748−2446ad, which spins 716 times per second[40]). Any small deviation from axial symmetry (a tiny "mountain" on the surface) will generate long duration periodic gravitational waves.[41] A number of potential mechanisms have been identified which could generate some "mountains" due to thermal, mechanic, or magnetic effects; accretion may also induce a break in axial symmetry.[42][43][44]
Another possible source of continuous waves in the Virgo detection range could be more exotic objects, such has dark matter candidates. Axions rotating around a black hole[42] or binary systems consisting of a primordial low-mass black hole and another compact object have in particular been suggested as potential sources. Some possible types of dark matter may be also detected by the interferometers directly, by interacting with optical elements of the device.[45]
Stochastic background
Several physical phenomena may be the source of a gravitational wave stochastic background, an additional source of noise of astrophysical origin. It represents a continuous source of gravitational waves, but unlike other continuous wave sources (like rotating neutron stars), it comes from large regions of the sky instead of a single location.[46]
The cosmic microwave background (CMB) is the earliest time of the Universe that can be observed in the electromagnetic spectrum. However, cosmological models predict the emission of gravitational waves generated instants after the Big Bang. Because gravitational waves interact very weakly with matter, detecting such background would give more insight in the cosmological evolution of our Universe.[47] In particular, it could provide evidence for inflation, from gravitational waves emitted either by the process of inflation itself (according to some theories)[48][49] or at the end of inflation.[50]
Moreover, Advanced Virgo may be able to detect an astrophysical background resulting from the superposition of all faint and distant sources emitting gravitational waves at all times, which would help to study the evolution of astrophysical sources and star formation. The most likely sources to contribute to the astrophysical background are binary neutron stars,[29] binary black holes,[51] or neutron star-black hole binaries. Other possible sources include supernovae and pulsars.[46]
Finally, cosmic strings may represent a source of gravitational wave background, whose detection could provide proof that cosmic strings actually exist.[52][53]
Exotic sources
Non-conventional, alternative models of compact objects have been proposed by physicists. Some examples of these models can be described within general relativity (quark and strange stars,[54] boson[55] and Proca stars, Kerr black holes with scalar and Proca hair), arise from some approaches to quantum gravity (cosmic strings,[56] fuzzballs,[57] gravastars[58]), or come from alternative theories of gravity (scalarised neutron stars or black holes, wormholes). Theoretically predicted exotic compact objects could now be detected and would help to elucidate the true nature of gravity or discover new forms of matter. Besides, completely unexpected phenomena may be observed, unveiling new physics.
Gravitational wave polarization
Gravitational waves are expected to have two "tensor" polarizations, nicknamed "plus" and "cross" due to their effects on a ring of particle (displayed in the figure below). A single gravitational wave is usually a superposition of these two polarizations, depending on the orientation of the source.
In addition, some theories of gravity allow for additional polarizations to exist: the two "vector" polarizations (x and y), and the two "scalar" polarizations ("breathing" and "longitudinal"). Detecting these additional polarizations could provide evidence for physics beyond general relativity.[59]
The polarizations can only be distinguished using several detectors; they could only be properly probed after Virgo was introduced, as the two LIGO detectors are almost co-aligned.[26] They can be measured from compact binary coalescences,[60][61] but also from the stochastic background[62] and continuous waves.[63] With the combination of the LIGO and Virgo detectors, it is possible to determine the presence or absence of the additional polarizations, but not their nature; a total of 5 independent detectors would be required to fully separate all the polarizations (except for the longitudinal and breathing polarizations, which cannot be distinguished from each other by detectors such as LIGO and Virgo[61]).[64]
- Effect of gravitational wave polarizations on a ring of particles
- Plus polarization
- Cross polarization
Lensed gravitational waves
General relativity predicts that a gravitational wave should be subject to gravitational lensing, just has light waves are; that is, the trajectory of a gravitational wave will be curved by the presence of a massive object (typically a galaxy or a galaxy cluster) near its path.[65] This can result in an increase in the amplitude of the wave, or even multiple observations of the event at different times, as we currently observe for the light of supernovae. Such events are predicted to be common enough to be detected by the current detectors in the near future.[66] Microlensing effects are also predicted.[67] Detecting a lensed event would allow for a very precise localization, as well as further tests of the speed of gravity and of the polarization.[65]
Cosmological measurements
Gravitational waves also provide a new way to measure some cosmological parameters, and in particular the Hubble parameter , which represents the rate of the expansion of the universe and whose value is currently disputed due to conflicting measurement from different methods. There are two main possibilities for measuring with gravitational waves in current detectors:
- Multi-messenger events with both a gravitational wave and an electromagnetic signal can be used, by measuring the distance with the gravitational wave signal and the recession speed with the electromagnetic wave (usually by identifying the galaxy in which the event took place), and applying Hubble's law. The main benefit here is that the distance measured from the gravitational waves does not rely on other measurements or assumptions, as is usually the case.[68]
- A statistical treatment can be applied to the observed population of binary black hole mergers (often called "dark sirens" in this context), constraining both their mass distribution and ; an external galaxy catalog can also be added to the analysis to improve the measurement.[69]
Testing general relativity
The measurement of gravitational wave signals offers a unique perspective for testing results from general relativity, as they are produced in environments where the gravitational field is very strong (e.g., near black holes). A number of tests of the predictions from general relativity can thus be made using the detected events. Such tests may uncover physics beyond general relativity, or possible issues in the models.[70]
- Looking for a residual signal in the data after subtracting models of the signal, which may indicate that some of the signal is not correctly modelled by general relativity.
- Checking that the signal from a merger satisfies some basic assumptions, such as verifying that the estimated parameters of the system are consistent across the different phases of the signal ("inspiral-merger-ringdown consistency test").[73]
- Introducing perturbations in the models for simulating gravitational waves to see if they fit the data.
- Investigating possible dispersion (absent from general relativity but not from alternative theories).[74]
- Analyzing the remnant of a merger, by measuring the post-merger phase of the signal ("ringdown") which is supposed to be fully determined by the mass and spin of the remnant. This enables testing the predictions for the energy lost to gravitational waves during the merger and the nature of the remnant object; some hypothetical objects may also feature "echoes" of the ringdown signal.
- Looking for non-standard polarizations (as seen above).
Instrument

Detection principle
In general relativity, a gravitational wave is a space-time perturbation which propagates at the speed of light. It thus slightly curves the space-time, which locally changes the light path. Mathematically speaking, if is the amplitude (assumed to be small) of the incoming gravitational wave and the length of the optical cavity in which the light is in circulation, the change of the optical path due to the gravitational wave is given by the formula:[75]
with being a geometrical factor which depends on the relative orientation between the cavity and the direction of propagation of the incoming gravitational wave. In other terms, the change in length is proportional to both to the length of the cavity and the amplitude of the gravitational wave.
Interferometer
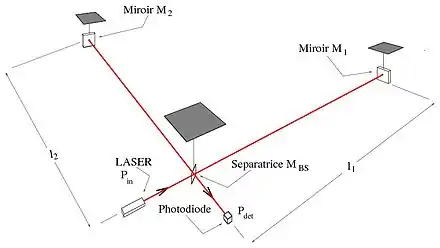
Virgo is a Michelson interferometer whose mirrors are suspended. A laser is divided into two beams by a beam splitter tilted by 45 degrees. The two beams propagate in the two perpendicular arms of the interferometer, are reflected by mirrors located at the end of the arms, and recombine on the beam splitter, generating interferences which are detected by a photodiode. An incoming gravitational wave changes the optical path of the laser beams in the arms, which then changes the interference pattern recorded by the photodiode.
This means the various mirrors of the interferometer must be "frozen" in position: when they move, the optical cavity length changes and so does the interference signal read at the instrument output port. The mirror positions relative to a reference and their alignment are monitored accurately in real time[76] with a precision better than the tenth of a nanometre for the lengths;[77] at the level of a few nanoradians for the angles. The more sensitive the detector, the narrower its optimal working point. Reaching that working point from an initial configuration in which the various mirrors are moving freely is a control system challenge; a complex series of steps is required to coordinate all the steerable parts of the interferometer. Once the working point is achieved, corrections are continuously applied in order to keep it in the optimal configuration.[78]
The signal induced by a potential gravitational wave is thus "embedded" in the light intensity variations detected at the interferometer output.[79] Yet, several external causes—globally denoted as noise—change the interference pattern perpetually and significantly. Should nothing be done to remove or mitigate them, the expected physical signals would be buried in noise and would then remain undetectable. The design of detectors like Virgo and LIGO thus requires a detailed inventory of all noise sources which could impact the measurement, allowing a strong and continuing effort to reduce them as much as possible.[80][77]
Using an interferometer rather than a single optical cavity allows one to enhance significantly the detector's sensitivity to gravitational waves. Indeed, in this configuration based on an interference measurement, the contributions from some experimental noises are strongly reduced: instead of being proportional to the length of the single cavity, they depend in that case on the length difference between the arms (so equal arm length cancels the noise). In addition, the interferometer configuration benefits from the differential effect induced by a gravitational wave in the plane transverse to its direction of propagation: when the length of an optical path changes by a quantity , the perpendicular optical path of same length changes by (same magnitude but opposite sign). And the interference at the output port of a Michelson interferometer depends on the difference of length between the two arms: the measured effect is hence amplified by a factor of 2 compared to a simple cavity.
The optimal working point of an interferometric detector of gravitational waves is slightly detuned from the "dark fringe", a configuration in which the two laser beams recombined on the beam splitter interfere in a destructive way: almost no light is detected at the output port.
Laser and injection system
The laser is the light source of the experiment. It must be powerful, while extremely stable in frequency and amplitude.[81] To meet all these (somewhat opposing) specifications, the beam starts from a very low power, yet very stable, laser.[82] The light from this laser passes through several amplifiers which enhance its power by a factor of 100. A 50 W output power was achieved for the last configuration of the initial Virgo detector, and later reached 100 W during the O3 run, following the Advanced Virgo upgrades; it is expected to be upgraded to 130 W at the beginning of the O4 run.[32] The original Virgo detector used a master-slave laser system, where a "master" laser is used to stabilize a high-powered "slave" laser; the master laser was a Nd:YAG laser, and the slave laser a Nd:YVO4 laser.[18] The retained solution for Advanced Virgo is to have a fiber laser with an amplification stage made of fibers as well, to improve the robustness of the system; in its final configuration, it is planned to coherently combine the light of two lasers in ordered to achieve the required power.[24][83] The wavelength of the laser is 1064 nanometres, both in the original and the Advanced Virgo configurations.[32]
This laser is then sent into the interferometer after passing through the injection system, which further ensures the stability of the beam, adjusts its shape and power, and positions it correctly for entering the interferometer. Key components of the injection system include the input mode cleaner (a 140-metre-long cavity allowing for cleaning the beam), a Faraday isolator preventing any light from returning to the laser, and a mode matching telescope, which adapts the size and position of the beam right before it enters the interferometer.[24]
Mirrors
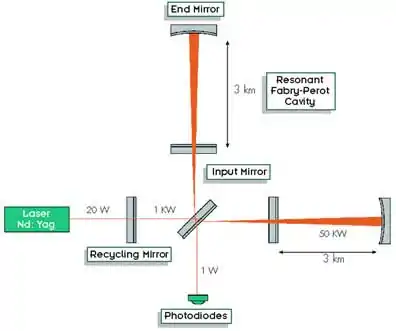
The large mirrors of the arm cavities are the most critical optics of the interferometer. They include the two end mirrors, located at the ends of the 3-km interferometer arms, and the two input mirrors, located near the beginning of the arms. Together, those mirrors make a resonant optical cavity in each arm, where the light bounces thousands of times before returning to the beam splitter, maximizing the effect of the signal on the laser path.[84] It also allows to increase the power of the light stored in the arms. These mirrors are non-standard pieces, made from state-of-the-art technologies. They are cylinders 35 cm in diameter and 20 cm thick,[24] made from the purest glass in the world.[85] The mirrors are polished to the atomic level in order to not diffuse (and hence lose) any light.[86] Finally, a reflective coating (a Bragg reflector made with ion beam sputtering, or IBS) is added. The mirrors located at the end of the arms reflect all incoming light; less than 0.002% of the light is lost at each reflection.[87]
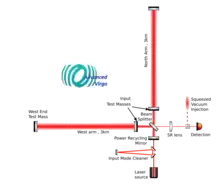
In addition, two other mirrors are present in the final design:
- The power recycling mirror, placed between the laser and the beam splitter. As most light is reflected toward the laser after returning to the beam splitter, this mirror re-injects this light back into the main interferometer, increasing the power in the arms.
- The signal recycling mirror (currently planned to be installed for the next run as part of the Advanced Virgo+ upgrades) re-injects part of the signal within the interferometer (currently, the transmission of this mirror is planned to be 40%), effectively forming another cavity. By making small adjustments to this signal recycling mirror, quantum noise can be reduced in part of the frequency band, while increasing it elsewhere, making it possible to tune the interferometer for certain frequencies. It is currently planned to use the "wideband" configuration, decreasing the noise at high and low frequencies but increasing it at intermediate frequencies. The decreased noise at high frequencies is of particular interest to study the signal from moments right before and after a merger.[32][11]

In order to mitigate the seismic noise which could propagate up to the mirrors, shaking them and hence obscuring potential gravitational wave signals, the large mirrors are suspended by a complex system. All of the main mirrors are suspended by four thin fibers made of silica[88] (glass) which are attached to a series of attenuators. This chain of suspension, called the "superattenuator", is close to 10 meters high and is also under vacuum.[89] The superattenuators do not only limit the disturbances on the mirrors, they also allow the mirror position and orientation to be precisely steered. The optical table where the injection optics used to shape the laser beam are located, such as the benches used for the light detection, are also suspended and under vacuum, in order to limit the seismic and acoustic noises. For Advanced Virgo, the whole instrumentation used to detect gravitational waves signals and to steer the interferometer (photodiodes, cameras, and the associated electronics) are also installed on several suspended benches, and under vacuum. This choice and the use of light traps (called baffles) inside the vacuum pipes prevent the residual seismic noise from being reintroduced into the dark port signals because of spurious reflections from diffused light.
Detection system
Part of the light circulating in the arm cavities is sent towards the detection system by the beam splitter. In its optimal configuration, the interferometer works close to the "dark fringe", meaning that very little light is sent towards the output; most of it is sent back to the input, to be collected by the power recycling mirror. A fraction of this light is reflected back by the signal recycling mirror, and the rest is set to be collected by the detection system. It first passes through the output mode cleaner, which further cleans the beam from any unwanted modes, before reaching the photodiodes, which measure the light amplitude. Both the output mode cleaner and the photodiodes are suspended and under vacuum.[23]
Starting with the O3 run, a vacuum squeezing system was introduced in order to reduce the shot noise, based on a dedicated optical device placed before the output mode cleaner. Squeezing designates a state where the quantum uncertainty of a specific quantity is decreased, always at the expense of increasing the uncertainty of another quantity. During the O3 run, frequency-independent squeezing was implemented, meaning that the squeezing is identical at all frequencies; this squeezing was used to reduce the shot noise and increase the radiation pressure noise, as the latter was not limiting the instrument's sensitivity.[90] It is currently planned that frequency-dependent squeezing will be installed, allowing to only reduce the shot noise at high frequency (where radiation pressure noise is not relevant), and reduce the radiation pressure noise at low frequencies (where shot noise is low). It requires the installation of an additional 285-metre-long cavity in order to produce the correct squeezed vacuum.[91][92] It has been estimated that the current squeezing setup has reduced the shot noise limit by 3.2 dB, resulting in an increase of the range of the detector by 5–8%.[93]
Infrastructure
Seen from the air, the Virgo detector has a characteristic "L" shape with its two 3-km-long perpendicular arms. The arm "tunnels" house vacuum pipes in which the laser beams are travelling under ultra-high vacuum.
Virgo is the largest ultra-high vacuum installation in Europe, with a total volume of 6,800 cubic meters.[94] The two 3-km arms are made of a long steel pipe 1.2m in diameter in which the target residual pressure is about 1 thousandth of a billionth of an atmosphere (improving by a factor 100 on the original Virgo level). Thus, the residual gas molecules (mainly hydrogen and water) are not disturbing the path of the laser beams.[24] Large gate valves are located at both ends of the arms so that work can be done in the mirror vacuum towers without breaking an arm's ultra-high vacuum. Indeed, both Virgo arms have been kept under vacuum since 2008.[95] The towers containing the mirrors and attenuators are themselves split in two sections with different pressures.[96] The tubes undergo a process called baking, where they are heated at 150°C in order to remove unwanted particles stuck on the surfaces; while the towers were also baked-out in the initial Virgo design, cryogenic traps are now used to prevent contamination.[24]
Due to the high power in the interferometer, the mirrors are susceptible to thermal effects due to the heating induced by the laser (despite having an extremely low absorption). These thermal effects can take the shape of a deformation of the surface due to dilation, or a change in the refractive index of the substrate; this results in power escaping from the interferometer and in perturbations of the signal. These two effects are accounted for by the thermal compensation system (TCS), which includes sensors called Hartmann wavefront sensors[97] (HWS), used to measure the optical aberration through an auxiliary light source, and two actuators: CO2 lasers, which selectively heat parts of the mirror to correct the defects, and ring heaters, which precisely adjust the radius of curvature of the mirror. The system also corrects the "cold defects", which are permanent defects introduced during the mirror manufacturing.[98][24] During the O3 run, the TCS was able to increase the power circulating inside the interferometer by 15%, and decrease the power leaving the interferometer by a factor of 2.[99]
Another important component is the system for controlling stray light, which refers to any light leaving the designated path of the interferometer, either by scattering on a surface or from unwanted reflection. The recombination of this stray light with the main beam of the interferometer can be a significant source of noise, and is often hard to track and to model. Most of the efforts to mitigate stray light are based on absorbing plates called "baffles", placed near the optics as well as within the tubes; additional precautions are needed to prevent the baffles from having an effect on the interferometer operation.[100][101][94]
Finally, the instrument requires an efficient data acquisition system. This system is in charge of managing the data measured at the output of the interferometer and from the many sensors present on the site, writing it in files, and distributing the files for data analysis. To this end, dedicated hardware and software have been developed in order to accommodate the specific needs of Virgo.[102]
Noise sources
Due to the precision required in the measurement, the Virgo detector is sensitive to a number of sources of noise which limit the precision of the measurement. Some of these sources correspond to large frequency ranges and limit the overall sensitivity of the detector,[77][94] such as:
- seismic noise (any ground motion from numerous sources, such as waves in the Mediterranean Sea, wind, or human activity like traffic), generally in the low frequencies up to about 10 Hertz (Hz)
- thermal noise of the mirrors and their suspension wires, corresponding to the agitation of the mirror/suspension from its own temperature, from a few tens to a few hundreds of Hz
- quantum noise, which includes the laser shot noise, corresponding to the fluctuation of the power received by the detectors and relevant above a few hundreds of Hz, and the radiation pressure noise, corresponding to the pressure applied by the laser on the mirror, which is relevant at low frequency
- Newtonian noise, caused by the variation of the gravity field which affects the position of the mirror, relevant below 20 Hz
In addition to these broad noise sources, a number of peaks are visible in the noise spectrum, related to specific noise sources. These notably include a line at 50 Hz (as well as harmonics at 100, 150, and 200 Hz), corresponding to the frequency of the European power grid; so-called "violin modes" at 300 Hz (and a number of harmonics), corresponding to the resonance frequency of the suspension fibers (which can vibrate at a specific frequency just as the strings of a violin do); and calibration lines, appearing when mirrors are moved for calibration.[103][104]
Additional noise sources may also have a short-term impact—bad weather or earthquakes may temporarily increase the noise level.[94]
Finally, a number of short-lived artifacts may appear in the data due to many possible instrumental issues; these are usually referred to as 'glitches'. It is estimated that about 20% of the detected events are impacted by glitches, requiring specific data processing methods to mitigate their impact.[105]
Detector sensitivity

A detector like Virgo is characterized by its sensitivity, a figure of merit providing information about the tiniest signal the instrument could detect—the smaller the value of the sensitivity, the better the detector. The sensitivity varies with frequency as each noise has its own frequency range.
The most common measure for the sensitivity of a gravitational wave detector is the "horizon distance", defined as the distance at which a binary neutron star with masses 1.4 M☉–1.4 M☉ (where M☉ is the solar mass) produces a signal-to-noise ratio of 8 in the detector. It is generally expressed in megaparsecs.[107] For instance, the range for Virgo during the O3 run was between 40 and 50 Mpc.[33] This range is only an indicator and does not represent a maximal range for the detector; signals from more massive sources will have a larger amplitude, and can thus be detected from further away.
Virgo is a wide band detector whose sensitivity ranges from a few Hz up to 10 kHz. Mathematically speaking, its sensitivity is characterized by its power spectrum which is computed in real time using the data recorded by the detector. The curve opposite shows an example of a Virgo amplitude spectrum density (the square root of the power spectrum) from 2011, plotted using log-log scale.
Calculations show that the detector sensitivity roughly scales as , where is the arm cavity length and the laser power on the beam splitter. To improve it, these two quantities must be increased. This is achieved by having long arms, using optical cavities inside the arm to maximize the exposition to the signal, and implementing power recycling to increase the power in the arms.[77][108]
Data analysis
An important part of the Virgo collaboration resources is dedicated to the development and deployment of data analysis software designed to process the output of the detector. Apart from the data acquisition software and the tools for distributing the data, this effort is mostly shared with members of the LIGO and KAGRA collaborations, as part of the LIGO-Virgo-KAGRA (LVK) collaboration.[109]
The data from the detector is initially only available to LVK members; segments of data around detected events are released at the time of publication of the related paper, and the full data is released after a proprietary period, currently lasting 18 months. During the third observing run (O3), this resulted in two separated data releases (O3a and O3b), corresponding to the first six months and last six months of the run respectively.[110] The data is then available for anyone on the Gravitational Wave Open Science Center (GWOSC) platform.[111][112]
Event detection pipelines
During the O3 run, five different pipelines were used to identify event candidates within the data. Four of them (GstLAL, PyCBC, MBTA, and SPIIR) were dedicated to the detection of compact binary coalescences (CBC, the only type of event detected so far), while the fifth one (cWB) was designed to detect any signal.[113]
All five pipelines have been used during the run ("online") as part of the low-latency alert system, and after the run ("offline") to spot events which may have been missed (except for SPIIR, which was only run online).[113]
The four CBC pipelines all rely on the concept of matched filtering, a technique which can be used to look for a known signal within noisy data in an optimal way. The major issue with using this technique to search for gravitational wave is that it requires some knowledge of what the signal looks like. Although reasonable models exist, the complexity of the equations governing the dynamics of a compact merger makes the generation of accurate waveforms difficult; the development of new waveforms is still an active field of research.[114][115] In addition, the sources cover a wide range of possible parameters (masses and spins of the two objects, sky location) which will yield different waveforms, instead of having one specific signal. This prompts the researchers to generate "template banks" containing a large amount of different waveforms corresponding to different parameters; a compromise has to be done between how tight the bank is (maximizing the number of detections) and the limited computational resources available to carry out the search with all the templates. How to generate such template banks efficiently is also an active field of research.[116]
Although the four searches use the same base technique, they all have different optimizations and specificities on how they handle the data. In particular, they use different techniques for estimating the significance of an event, for discriminating between real events and glitches, and for combining the data from the different detectors; they also use different template banks.
The cWB (coherent wave burst) pipeline uses a completely separate approach: it works by grouping the streams of the different detectors and carrying a joint analysis of the data to look for coherent signals among the different detectors. Although its sensitivity for binary mergers is less than the dedicated CBC pipelines, its strength lies in being able to detect signals from any kind of sources, as it does not require any assumption on the shape of the signal (which is why it often referred to as an "unmodeled" search).[117]
Low-latency
The low-latency system is designed to produce alerts for astronomers when gravitational events are detected, with the hope that an electromagnetic counterpart can be observed. This is achieved by centralizing the event candidates from the different analysis pipelines in the gravitational-wave candidate event database (GraceDB),[118] from which the data is processed. If an event is deemed significant enough, a rapid sky localization is produced and preliminary alerts are sent autonomously within the span of a few minutes; after a more precise evaluation of the source parameters, as well as human vetting from the rapid response team, a new alert or a retraction notice is sent within a day.[119] The alerts are sent through the GCN, which also centralizes alerts from gamma-ray and neutrino telescopes, as well as SciMMA.[120][121] A total of 78 alerts were sent during the O3 run, of which 23 were later retracted.[113]
Parameter estimation
After an event has been detected by one of the event detection pipelines, a deeper analysis is performed in order to get a more precise estimation of the parameters of the source and the measurement uncertainty. During the O3 run, this was carried out using several different pipelines, including Bilby and RIFT. These pipelines employ Bayesian methods to quantify the uncertainty, including MCMC and nested sampling.[113]
Search for counterparts
While many astronomers try to follow-up the low-latency alerts from gravitational wave detectors, the reverse also exists: electromagnetic events expected to have an associated gravitational wave emission are subjected to a deeper search. One of the prime targets for these are gamma-ray bursts; these are thought to be associated with supernovae ("long" bursts, lasting more than 2 seconds) and with compact binary coalescenses involving neutron stars ("short" bursts).[122] The merger of two neutron stars in particular has been confirmed to be associated with both a gamma-ray burst and gravitational waves with the GW170817 event.[26]
Searches targeted toward gamma-ray bursts have been performed on the past runs using the pyGRB pipeline[123] for CBC, using methods similar to the regular searches, but centered around the time of the bursts and targeting only the sky area found by gamma-ray observatories. An unmodelled search was also carried out using the X-pipeline package, in a similar fashion as regular unmodelled searches.[124][122]
In addition to these searches, several pipelines are looking for coincidences between alerts from gravitational waves and alerts from other detectors. In particular, the RAVEN pipeline is part of the low-latency infrastructure and analyzes the coincidence with gamma-ray burst events and other sources.[125] The LLAMA pipeline is also dedicated to identifying such coincidences with neutrino events, predominantly from IceCube.[126]
Continuous wave searches
Searches dedicated to periodic gravitational waves—such as the ones generated by rapidly rotating neutron stars—are generally referred to as continuous wave searches. These can be divided in three categories: all-sky searches, which look for unknown signals from any direction, directed searches, which aim for objects with known positions but unknown frequency, and targeted searches, which hunt for signals from sources where both the position and the frequency are known. The directed and targeted searches are motivated by the fact that all-sky searches are extremely computationally expensive, and thus require trade-offs that limit their sensitivity.[42][44]
The principal challenge in continuous wave search is that the signal is much weaker than current detected transients, meaning that one must observe a long time period to accumulate enough data to detect it, as the signal-to-noise ratio scales with the square root of the observing time (intuitively, the signal will add up over the observing duration while the noise will not).[127] The issue is that over such long periods of time, the frequency from the source will evolve, and the motion of the Earth around the Sun will affect the frequency via the Doppler effect. This greatly increases the computational cost of the search, even more so when the frequency is unknown. Although there are mitigation strategies, such as semi-coherent searches, where the analysis is performed separately on segments from the data rather than the full data, these result in a loss of sensitivity.[42] Other approaches include cross-correlation, inspired by stochastic wave searches, which takes advantage of having multiple detectors to look for a correlated signal in a pair of detectors.[128]
Stochastic wave searches
The stochastic gravitational wave background is another target for data analysis teams. By definition, it can be seen as a source of noise in the detectors; the main challenge is to separate it from the other sources of noise, and measure its power spectral density. The easiest method for solving this issue is to look for correlations between two detectors; the idea being that the noise related to the gravitational wave background will be identical in both detectors, while the instrumental noise will not be correlated across the detectors. Another possible approach would be to look for excess power not accounted by other noise sources; however, this proves impractical for current interferometers (including Virgo) as the noise is not known well enough compared to the expected power of the background.[129] Only searches based on cross-correlation between detectors are currently in use.[130]
This kind of search must also account for factors such as the detectors antenna pattern, the motion of the Earth, and the distance between the detectors. Assumptions also have to be made on some properties of the background; it is common to assume that it is Gaussian and isotropic, but searches for anisotropic, non-Gaussian, and more exotic backgrounds also exist.[129]
Gravitational wave properties searches
A number of software have been developed to investigate the physics surrounding gravitational waves. These analyses are generally performed offline (after the run), and often rely on the results from the other searches (currently mostly CBC searches).
Several analyses are performed to look for events observed multiple time due to lensing, first by trying to match all the known events together, and then by performing a joint analysis for the most promising pair of events; these analyses have been performed using LALInference and HANABI softwares. Additional searches for events which may have been missed by the regular CBC searches are also performed, by reusing the existing CBC pipelines.[65]
Software designed for estimating the Hubble constant has also been developed. The gwcosmo pipeline performs a Bayesian analysis to determine a distribution of the possible values of the constant, both using "dark sirens" (CBC events without electromagnetic counterpart), which can be correlated with a galaxy catalog, and events with an electromagnetic counterpart for which a direct estimation can be made based on the distance measured with gravitational waves and the identified host galaxy.[131][132] This requires assuming a specific population of black holes, which may be a significant source of bias; recent analyses have been trying to circumvent this issue by fitting both the population and the Hubble constant simultaneously.[133]
Scientific results

The first detection of a gravitational signal by Virgo took place at the beginning of the second observing run (O2), as Virgo was absent from the first observing run. The event, named GW170814, was a coalescence between two black holes, and also the first event to be detected by three different detectors, allowing for its localization to be greatly improved compared to the events from the first observing run. It also allowed for the first conclusive measure of gravitational wave polarizations, providing evidence against the existence of polarizations other than the ones predicted by general relativity.[26]
It was soon followed by the more famous GW170817, first merger of two neutron stars detected by the gravitational wave network, and as of January 2023 the only event with a confirmed detection of an electromagnetic counterpart, both in gamma rays and in optical telescopes, and later in the radio and x-ray domains. While no signal was observed in Virgo, this absence was crucial to put tighter constraints on the localization of the event.[28] This event had tremendous repercussions in the astronomical community, involving more than 4000 astronomers,[134] improving the understanding of neutron star mergers,[135] and putting very tight constraints on the speed of gravity.[136]
Several searches for continuous gravitational waves have been performed on data from the past runs. On the O3 run, these include an all-sky search,[137] targeted searches toward Scorpius X-1[138] and a number of known pulsars (including the Crab and Vela pulsars),[139][140] and directed search towards the supernova remnants Cassiopeia A and Vela Jr.[141] and the galactic center.[142] While none of the sources managed to identify a signal, this allowed upper limits to be set on some parameters; in particular, it was found that the deviation from perfect spinning balls for close known pulsars is at most of the order of 1 mm.[137]
Virgo was included in the latest search for a gravitational wave background along with LIGO, combining the results of O3 with the ones from the O1 and O2 runs (which only used LIGO data). No stochastic background was observed, improving previous constraints on the energy of the background by an order of magnitude.[143]
Constraints on the Hubble constant have also been obtained; the current best estimate is 68+12
-8 km s−1 Mpc−1, combining results from binary black holes and from the GW170817 event. This result is coherent with other estimates of the constant, but not precise enough to resolve the tension regarding its exact value.[144]
Outreach
The Virgo collaboration participates in a number of activities promoting communication and education on gravitational waves towards the general public.[145] This includes a wide variety of activities, such as:
- participation in numerous science festivals[146][147][148]
- public lectures and courses about Virgo activities, including toward school classes[145]
- participation in art exhibitions, such as "The Rhythm of Space" at the Museo della Grafica in Pisa,[149] or "On Air" at the Palais de Tokyo[150]
- organize guided tours of the Virgo facilities for schools, universities, and the general public[151]
- involvement in activities promoting gender equality in science[152]
Gallery
- Overview of the Virgo site
- Aerial view of the Virgo detector
- View of the 3-km-long Virgo north arm
- The Virgo site with, in the foreground, the building which hosts the detector control room and the local computer center.
- The Virgo central building which hosts the laser and the beamsplitter mirror.
- View of the 3-km-long Virgo west arm (right pipe). The tube on the left, which is 150 metres long, hosts the mode-cleaner cavity which is used to spatially filter the laser beam.
References
- "Our mission". www.ego-gw.it. European Gravitational Observatory. Retrieved 2021-10-04.
- "The Virgo Collaboration". virgo-gw.eu. The Virgo Collaboration. 2021-02-18. Retrieved 2021-10-04.
- "LIGO-M060038-v2: Memorandum of Understanding Between VIRGO and LIGO". LIGO. 2014. Retrieved 2016-02-13.
- "Communique de presse – Le CNRS signe l'accord franco-italien de création du consortium EGO European Gravitational Observatory". Cnrs.fr. Archived from the original on 2016-03-05. Retrieved 2016-02-11.
- "Gravitational wave detectors prepare for next observing run – Virgo". www.virgo-gw.eu. Retrieved 2023-05-04.
- "The Virgo Institutions". virgo-gw.eu. The Virgo Collaboration. Retrieved 2021-10-04.
- "Scientific Collaboration – Virgo". www.virgo-gw.eu. Retrieved 2023-03-31.
- "LIGO Scientific Collaboration - Learn about the LSC". www.ligo.org. Retrieved 2023-03-31.
- Einstein, Albert (1916-01-01). "Näherungsweise Integration der Feldgleichungen der Gravitation". Sitzungsberichte der Königlich Preussischen Akademie der Wissenschaften: 688–696. Bibcode:1916SPAW.......688E.
- Weber, J. (1968-06-03). "Gravitational-Wave-Detector Events". Physical Review Letters. 20 (23): 1307–1308. Bibcode:1968PhRvL..20.1307W. doi:10.1103/PhysRevLett.20.1307.
- Bersanetti, Diego; Patricelli, Barbara; Piccinni, Ornella Juliana; Piergiovanni, Francesco; Salemi, Francesco; Sequino, Valeria (August 2021). "Advanced Virgo: Status of the Detector, Latest Results and Future Prospects". Universe. 7 (9): 322. Bibcode:2021Univ....7..322B. doi:10.3390/universe7090322. ISSN 2218-1997.
- Giazotto, Adalberto (2018). La musica nascosta dell'universo: La mia vita a caccia delle onde gravitazionali (in Italian). Einaudi. ASIN B07FY52PGV. Bibcode:2018lmnd.book.....G.
- Giazotto, Adalberto; Milano, Leopoldo; Bordoni, Franco; Brillet, Alain; Tourrenc (1987-05-12). Proposta di Antenna interferometrica a grande base per la ricerca di Onde Gravitazionali (PDF) (Technical report).
- Caron, B.; Dominjon, A.; Drezen, C.; Flaminio, R.; Grave, X.; Marion, F.; Massonnet, L.; Mehmel, C.; Morand, R.; Mours, B.; Yvert, M.; Babusci, D.; Giordano, G.; Matone, G.; Mackowski, J. -M. (1996-05-01). "Status of the VIRGO experiment". Nuclear Physics B - Proceedings Supplements. Proceedings of the Fourth International Workshop on Theoretical and Phenomenological Aspects of Underground Physics. 48 (1): 107–109. Bibcode:1996NuPhS..48..107C. doi:10.1016/0920-5632(96)00220-4. ISSN 0920-5632.
- J.M. Weisberg and J.H. Taylor (2004). "Relativistic Binary Pulsar B1913+16: Thirty Years of Observations and Analysis". ASP Conference Series. 328: 25. arXiv:astro-ph/0407149. Bibcode:2005ASPC..328...25W.
- Riles, K. (2013). "Gravitational Waves: Sources, Detectors and Searches". Progress in Particle and Nuclear Physics. 68: 1–54. arXiv:1209.0667. Bibcode:2013PrPNP..68....1R. doi:10.1016/j.ppnp.2012.08.001. S2CID 56407863.
- B.S. Sathyaprakash and Bernard F. Schutz (2009). "Physics, Astrophysics and Cosmology with Gravitational Waves". Living Reviews in Relativity. 12 (1): 2. arXiv:0903.0338. Bibcode:2009LRR....12....2S. doi:10.12942/lrr-2009-2. PMC 5255530. PMID 28163611. Archived from the original on 2016-03-04. Retrieved 2016-02-11.
- Acernese, F.; Amico, P.; Al-Shourbagy, M.; Aoudia, S.; Avino, S.; et al. (August 2004). "The status of VIRGO". 5th Rencontres du Vietnam Particle Physics and Astrophysics. Hanoi, Vietnam: 1–6 – via HAL.
- "Ondes gravitationnelles : Virgo entre dans sa phase d'exploitation scientifique – Communiqués et dossiers de presse". Cnrs.fr. Retrieved 2016-02-11.
- Accadia, T.; Acernese, F.; Alshourbagy, M.; Amico, P.; Antonucci, F.; Aoudia, S.; Arnaud, N.; Arnault, C.; Arun, K. G.; Astone, P.; Avino, S.; Babusci, D.; Ballardin, G.; Barone, F.; Barrand, G.; Barsotti, L.; Barsuglia, M.; Basti, A.; Bauer, Th S.; Beauville, F.; Bebronne, M.; Bejger, M.; Beker, M. G.; Bellachia, F.; Belletoile, A.; Beney, J. L.; Bernardini, M.; Bigotta, S.; Bilhaut, R.; et al. (2012-03-29). "Virgo: a laser interferometer to detect gravitational waves – IOPscience". Journal of Instrumentation. 7 (3): P03012. Bibcode:2012JInst...7.3012A. doi:10.1088/1748-0221/7/03/P03012.
- Lorenzini, Matteo (April 2010). "The monolithic suspension for the Virgo interferometer". Classical and Quantum Gravity. 27 (8). doi:10.1088/0264-9381/27/8/084021.
- The Virgo Collaboration (2011). "Status of the Virgo project" (PDF). Classical and Quantum Gravity. 28 (11): 114002. Bibcode:2011CQGra..28k4002A. doi:10.1088/0264-9381/28/11/114002. S2CID 59369141.
- Acernese, F.; Agathos, M.; Agatsuma, K.; Aisa, D.; Allemandou, N.; Allocca, A.; Amarni, J.; Astone, P.; Balestri, G.; Ballardin, G.; Barone, F.; Baronick, J-P; Barsuglia, M.; Basti, A.; Basti, F.; Bauer, Th S.; Bavigadda, V.; Bejger, M.; Beker, M. G.; Belczynski, C.; Bersanetti, D.; Bertolini, A.; Bitossi, M.; Bizouard, M. A.; Bloemen, S.; Blom, M.; Boer, M.; Bogaert, G.; Bondi, D.; et al. (2015). "Advanced Virgo: A second-generation interferometric gravitational wave detector". Classical and Quantum Gravity. 32 (2): 024001. arXiv:1408.3978. Bibcode:2015CQGra..32b4001A. doi:10.1088/0264-9381/32/2/024001. S2CID 20640558.
- Many authors of the Virgo Collaboration (13 April 2012). Advanced Virgo Technical Design Report VIR–0128A–12 (PDF).
- Nicolas Arnaud: Status of the Advanced LIGO and Advanced Virgo detectors
- Abbott, B. P.; Abbott, R.; Abbott, T. D.; Acernese, F.; Ackley, K.; Adams, C.; Adams, T.; Addesso, P.; Adhikari, R. X.; Adya, V. B.; Affeldt, C.; Afrough, M.; Agarwal, B.; Agathos, M.; Agatsuma, K. (2017-10-06). "GW170814: A Three-Detector Observation of Gravitational Waves from a Binary Black Hole Coalescence". Physical Review Letters. 119 (14): 141101. arXiv:1709.09660. Bibcode:2017PhRvL.119n1101A. doi:10.1103/PhysRevLett.119.141101. ISSN 0031-9007. PMID 29053306. S2CID 46829350.
- "European detector spots its first gravitational wave". 27 September 2017. Retrieved 27 September 2017.
- Abbott, B. P.; Abbott, R.; Abbott, T. D.; Acernese, F.; Ackley, K.; Adams, C.; Adams, T.; Addesso, P.; Adhikari, R. X.; Adya, V. B.; Affeldt, C.; Afrough, M.; Agarwal, B.; Agathos, M.; Agatsuma, K. (2017-10-16). "Multi-messenger Observations of a Binary Neutron Star Merger". The Astrophysical Journal. 848 (2): L12. arXiv:1710.05833. Bibcode:2017ApJ...848L..12A. doi:10.3847/2041-8213/aa91c9. ISSN 2041-8213. S2CID 217162243.
- LIGO Scientific Collaboration and Virgo Collaboration; Abbott, B. P.; Abbott, R.; Abbott, T. D.; Acernese, F.; Ackley, K.; Adams, C.; Adams, T.; Addesso, P.; Adhikari, R. X.; Adya, V. B.; Affeldt, C.; Afrough, M.; Agarwal, B.; Agathos, M. (2018-02-28). "GW170817: Implications for the Stochastic Gravitational-Wave Background from Compact Binary Coalescences". Physical Review Letters. 120 (9): 091101. arXiv:1710.05837. Bibcode:2018PhRvL.120i1101A. doi:10.1103/PhysRevLett.120.091101. PMID 29547330. S2CID 3889124.
- Diego Bersanetti: Status of the Virgo gravitational-wave detector and the O3 Observing Run, EPS-HEP2019
- "LIGO Suspends Third Observing Run (O3)". LIGO Lab | Caltech. Retrieved 2023-04-16.
- Flaminio, Raffaele (2020-12-13). Marshall, Heather K.; Spyromilio, Jason; Usuda, Tomonori (eds.). "Status and plans of the Virgo gravitational wave detector". Ground-based and Airborne Telescopes VIII. SPIE. 11445: 205–214. Bibcode:2020SPIE11445E..11F. doi:10.1117/12.2565418. ISBN 9781510636774. S2CID 230549331.
- "IGWN | Observing Plans". observing.docs.ligo.org. Retrieved 2023-02-20.
- "Virgo postpones entry into O4 observing run – Virgo". www.virgo-gw.eu. Retrieved 2023-05-13.
- The Virgo Collaboration (2022-05-31). Virgo nEXT: beyond the AdV+ project - A concept study (PDF) (Technical report).
- "Astrophysical Sources of Gravitational Waves – Virgo". www.virgo-gw.eu. Retrieved 2023-03-31.
- "LIGO Scientific Collaboration - The science of LSC research". www.ligo.org. Retrieved 2023-03-31.
- Kotake, Kei (2013-04-01). "Multiple physical elements to determine the gravitational-wave signatures of core-collapse supernovae". Comptes Rendus Physique. 14 (4): 318–351. arXiv:1110.5107. Bibcode:2013CRPhy..14..318K. doi:10.1016/j.crhy.2013.01.008. ISSN 1631-0705. S2CID 119112669.
- Kotake, Kei; Takiwaki, Tomoya; Suwa, Yudai; Iwakami Nakano, Wakana; Kawagoe, Shio; Masada, Youhei; Fujimoto, Shin-ichiro (2012-11-07). "Multimessengers from Core-Collapse Supernovae: Multidimensionality as a Key to Bridge Theory and Observation". Advances in Astronomy. 2012: e428757. arXiv:1204.2330. Bibcode:2012AdAst2012E..39K. doi:10.1155/2012/428757. ISSN 1687-7969.
- Hessels, Jason W. T.; Ransom, Scott M.; Stairs, Ingrid H.; Freire, Paulo C. C.; Kaspi, Victoria M.; Camilo, Fernando (2006-03-31). "A Radio Pulsar Spinning at 716 Hz". Science. 311 (5769): 1901–1904. arXiv:astro-ph/0601337. Bibcode:2006Sci...311.1901H. doi:10.1126/science.1123430. ISSN 0036-8075. PMID 16410486. S2CID 14945340.
- Aasi, J.; Abadie, J.; Abbott, B. P.; Abbott, R.; Abbott, T.; Abernathy, M. R.; Accadia, T.; Acernese, F.; Adams, C.; Adams, T.; Adhikari, R. X.; Affeldt, C.; Agathos, M.; Aggarwal, N.; Aguiar, O. D. (2014-04-20). "Gravitational Waves from Known Pulsars: Results from the Initial Detector Era". The Astrophysical Journal. 785 (2): 119. arXiv:1309.4027. Bibcode:2014ApJ...785..119A. doi:10.1088/0004-637X/785/2/119. hdl:1721.1/92734. ISSN 0004-637X. S2CID 215729501.
- Riles, Keith (2022-06-13). "Searches for Continuous-Wave Gravitational Radiation". arXiv:2206.06447 [astro-ph.HE].
- Sieniawska, Magdalena; Bejger, Michał (November 2019). "Continuous Gravitational Waves from Neutron Stars: Current Status and Prospects". Universe. 5 (11): 217. arXiv:1909.12600. Bibcode:2019Univ....5..217S. doi:10.3390/universe5110217. ISSN 2218-1997.
- Piccinni, Ornella Juliana (June 2022). "Status and Perspectives of Continuous Gravitational Wave Searches". Galaxies. 10 (3): 72. arXiv:2202.01088. Bibcode:2022Galax..10...72P. doi:10.3390/galaxies10030072. ISSN 2075-4434.
- LIGO Scientific Collaboration, Virgo Collaboration, and KAGRA Collaboration; Abbott, R.; Abbott, T. D.; Acernese, F.; Ackley, K.; Adams, C.; Adhikari, N.; Adhikari, R. X.; Adya, V. B.; Affeldt, C.; Agarwal, D.; Agathos, M.; Agatsuma, K.; Aggarwal, N.; Aguiar, O. D. (2022-03-31). "Constraints on dark photon dark matter using data from LIGO's and Virgo's third observing run". Physical Review D. 105 (6): 063030. arXiv:2105.13085. Bibcode:2022PhRvD.105f3030A. doi:10.1103/PhysRevD.105.063030. S2CID 235212543.
- Christensen, Nelson (2019-01-01). "Stochastic gravitational wave backgrounds". Reports on Progress in Physics. 82 (1): 016903. arXiv:1811.08797. Bibcode:2019RPPh...82a6903C. doi:10.1088/1361-6633/aae6b5. ISSN 0034-4885. PMID 30462612. S2CID 53712558.
- Bar-Kana, Rennan (1994-07-15). "Limits on direct detection of gravitational waves". Physical Review D. 50 (2): 1157–1160. arXiv:astro-ph/9401050. Bibcode:1994PhRvD..50.1157B. doi:10.1103/PhysRevD.50.1157. PMID 10017813. S2CID 17756178.
- Lopez, Alejandro; Freese, Katherine (2015-01-28). "First test of high frequency Gravity Waves from inflation using Advanced LIGO". Journal of Cosmology and Astroparticle Physics. 2015 (1): 037. arXiv:1305.5855. Bibcode:2015JCAP...01..037L. doi:10.1088/1475-7516/2015/01/037. ISSN 1475-7516. S2CID 118722983.
- Barnaby, Neil; Pajer, Enrico; Peloso, Marco (2012-01-23). "Gauge field production in axion inflation: Consequences for monodromy, non-Gaussianity in the CMB, and gravitational waves at interferometers". Physical Review D. 85 (2): 023525. arXiv:1110.3327. Bibcode:2012PhRvD..85b3525B. doi:10.1103/PhysRevD.85.023525. S2CID 119269863.
- Easther, Richard; Giblin, John T.; Lim, Eugene A. (2007-11-26). "Gravitational Wave Production at the End of Inflation". Physical Review Letters. 99 (22): 221301. arXiv:astro-ph/0612294. Bibcode:2007PhRvL..99v1301E. doi:10.1103/PhysRevLett.99.221301. PMID 18233276. S2CID 43736564.
- LIGO Scientific Collaboration and Virgo Collaboration; Abbott, B. P.; Abbott, R.; Abbott, T. D.; Abernathy, M. R.; Acernese, F.; Ackley, K.; Adams, C.; Adams, T.; Addesso, P.; Adhikari, R. X.; Adya, V. B.; Affeldt, C.; Agathos, M.; Agatsuma, K. (2016-03-31). "GW150914: Implications for the Stochastic Gravitational-Wave Background from Binary Black Holes". Physical Review Letters. 116 (13): 131102. arXiv:1602.03847. Bibcode:2016PhRvL.116m1102A. doi:10.1103/PhysRevLett.116.131102. PMID 27081965. S2CID 216147156.
- Chang, Chia-Feng; Cui, Yanou (2022-03-17). "Gravitational waves from global cosmic strings and cosmic archaeology". Journal of High Energy Physics. 2022 (3): 114. arXiv:2106.09746. Bibcode:2022JHEP...03..114C. doi:10.1007/JHEP03(2022)114. ISSN 1029-8479. S2CID 235485257.
- Abbott, R.; Abbott, T. D.; Abraham, S.; Acernese, F.; Ackley, K.; Adams, A.; Adams, C.; Adhikari, R. X.; Adya, V. B.; Affeldt, C.; Agarwal, D.; Agathos, M.; Agatsuma, K.; Aggarwal, N.; Aguiar, O. D. (2021-06-16). "Constraints on Cosmic Strings Using Data from the Third Advanced LIGO–Virgo Observing Run". Physical Review Letters. 126 (24): 241102. arXiv:2101.12248. Bibcode:2021PhRvL.126x1102A. doi:10.1103/PhysRevLett.126.241102. hdl:1721.1/139689.2. ISSN 0031-9007. PMID 34213926. S2CID 231728406.
- Wang, Xu; Huang, Yong-Feng; Li, Bing (2021-09-30). "Searching For Strange Quark Planets". arXiv:2109.15161.
{{cite journal}}
: Cite journal requires|journal=
(help) - Pacilio, Costantino; Vaglio, Massimo; Maselli, Andrea; Pani, Paolo (2020-10-05). "Gravitational-wave detectors as particle-physics laboratories: Constraining scalar interactions with a coherent inspiral model of boson-star binaries". Physical Review D. 102 (8): 083002. arXiv:2007.05264. Bibcode:2020PhRvD.102h3002P. doi:10.1103/PhysRevD.102.083002. ISSN 2470-0010. S2CID 222129943.
- Auclair, Pierre; Blasi, Simone; Brdar, Vedran; Schmitz, Kai (2023). "Gravitational waves from current-carrying cosmic strings". Journal of Cosmology and Astroparticle Physics. 2023 (4): 009. arXiv:2207.03510. Bibcode:2023JCAP...04..009A. doi:10.1088/1475-7516/2023/04/009. S2CID 250408251.
- Mayerson, Daniel R. (2020-11-25). "Fuzzballs and observations". General Relativity and Gravitation. 52 (12): 115. arXiv:2010.09736. Bibcode:2020GReGr..52..115M. doi:10.1007/s10714-020-02769-w. ISSN 0001-7701. S2CID 224803627.
- Wang, Yu-Tong; Zhang, Jun; Piao, Yun-Song (2019-08-10). "Primordial gravastar from inflation". Physics Letters B. 795: 314–318. arXiv:1810.04885. Bibcode:2019PhLB..795..314W. doi:10.1016/j.physletb.2019.06.036. ISSN 0370-2693. S2CID 118970977.
- Eardley, Douglas M.; Lee, David L.; Lightman, Alan P.; Wagoner, Robert V.; Will, Clifford M. (1973-04-30). "Gravitational-Wave Observations as a Tool for Testing Relativistic Gravity". Physical Review Letters. 30 (18): 884–886. Bibcode:1973PhRvL..30..884E. doi:10.1103/PhysRevLett.30.884. hdl:2060/19730012613. S2CID 120335306.
- Takeda, Hiroki; Nishizawa, Atsushi; Michimura, Yuta; Nagano, Koji; Komori, Kentaro; Ando, Masaki; Hayama, Kazuhiro (2018-07-12). "Polarization test of gravitational waves from compact binary coalescences". Physical Review D. 98 (2): 022008. arXiv:1806.02182. Bibcode:2018PhRvD..98b2008T. doi:10.1103/PhysRevD.98.022008. S2CID 119234628.
- Isi, Maximiliano; Weinstein, Alan J. (2017-10-10). "Probing gravitational wave polarizations with signals from compact binary coalescences". arXiv:1710.03794 [gr-qc].
- Callister, Thomas; Biscoveanu, A. Sylvia; Christensen, Nelson; Isi, Maximiliano; Matas, Andrew; Minazzoli, Olivier; Regimbau, Tania; Sakellariadou, Mairi; Tasson, Jay; Thrane, Eric (2017-12-07). "Polarization-Based Tests of Gravity with the Stochastic Gravitational-Wave Background". Physical Review X. 7 (4): 041058. arXiv:1704.08373. Bibcode:2017PhRvX...7d1058C. doi:10.1103/PhysRevX.7.041058. S2CID 118992565.
- Isi, Maximiliano; Pitkin, Matthew; Weinstein, Alan J. (2017-08-15). "Probing dynamical gravity with the polarization of continuous gravitational waves". Physical Review D. 96 (4): 042001. arXiv:1703.07530. Bibcode:2017PhRvD..96d2001I. doi:10.1103/PhysRevD.96.042001. S2CID 3674818.
- Chatziioannou, Katerina; Yunes, Nicolás; Cornish, Neil (2012-07-23). "Model-independent test of general relativity: An extended post-Einsteinian framework with complete polarization content". Physical Review D. 86 (2): 022004. arXiv:1204.2585. Bibcode:2012PhRvD..86b2004C. doi:10.1103/PhysRevD.86.022004. S2CID 118890287.
- Abbott, R.; et al. (2021). "Search for Lensing Signatures in the Gravitational-Wave Observations from the First Half of LIGO–Virgo's Third Observing Run". The Astrophysical Journal. 923 (1): 14. arXiv:2105.06384. Bibcode:2021ApJ...923...14A. doi:10.3847/1538-4357/ac23db. S2CID 234482851.
- Li, Shun-Sheng; Mao, Shude; Zhao, Yuetong; Lu, Youjun (2018-05-11). "Gravitational lensing of gravitational waves: A statistical perspective". Monthly Notices of the Royal Astronomical Society. 476 (2): 2220–2229. arXiv:1802.05089. doi:10.1093/mnras/sty411. ISSN 0035-8711.
- Mishra, Anuj; Meena, Ashish Kumar; More, Anupreeta; Bose, Sukanta; Bagla, J. S. (2021-10-26). "Gravitational Lensing of Gravitational Waves: Effect of Microlens Population in Lensing Galaxies". Monthly Notices of the Royal Astronomical Society. 508 (4): 4869–4886. arXiv:2102.03946. doi:10.1093/mnras/stab2875. ISSN 0035-8711.
- Abbott, B. P.; Abbott, R.; Abbott, T. D.; Acernese, F.; Ackley, K.; Adams, C.; Adams, T.; Addesso, P.; Adhikari, R. X.; Adya, V. B.; Affeldt, C.; Afrough, M.; Agarwal, B.; Agathos, M.; Agatsuma, K. (November 2017). "A gravitational-wave standard siren measurement of the Hubble constant". Nature. 551 (7678): 85–88. arXiv:1710.05835. Bibcode:2017Natur.551...85A. doi:10.1038/nature24471. ISSN 1476-4687. PMID 29094696. S2CID 205261622.
- The LIGO Scientific Collaboration; the Virgo Collaboration; the KAGRA Collaboration; Abbott, R.; Abe, H.; Acernese, F.; Ackley, K.; Adhikari, N.; Adhikari, R. X.; Adkins, V. K.; Adya, V. B.; Affeldt, C.; Agarwal, D.; Agathos, M.; Agatsuma, K. (2021-11-19). "Constraints on the cosmic expansion history from GWTC-3". arXiv:2111.03604 [astro-ph.CO].
- Krishnendu, N. V.; Ohme, Frank (December 2021). "Testing General Relativity with Gravitational Waves: An Overview". Universe. 7 (12): 497. arXiv:2201.05418. Bibcode:2021Univ....7..497K. doi:10.3390/universe7120497. ISSN 2218-1997.
- Van Den Broeck, Chris (2014), Ashtekar, Abhay; Petkov, Vesselin (eds.), "Probing Dynamical Spacetimes with Gravitational Waves", Springer Handbook of Spacetime, Berlin, Heidelberg: Springer, pp. 589–613, doi:10.1007/978-3-642-41992-8_27, ISBN 978-3-642-41992-8, retrieved 2023-04-23
- LIGO Scientific Collaboration and Virgo Collaboration; Abbott, R.; Abbott, T. D.; Abraham, S.; Acernese, F.; Ackley, K.; Adams, A.; Adams, C.; Adhikari, R. X.; Adya, V. B.; Affeldt, C.; Agathos, M.; Agatsuma, K.; Aggarwal, N.; Aguiar, O. D. (2021-06-15). "Tests of general relativity with binary black holes from the second LIGO-Virgo gravitational-wave transient catalog". Physical Review D. 103 (12): 122002. arXiv:2010.14529. Bibcode:2021PhRvD.103l2002A. doi:10.1103/PhysRevD.103.122002. hdl:1721.1/139692. S2CID 225094618.
- Ghosh, Abhirup; Johnson-McDaniel, Nathan K; Ghosh, Archisman; Mishra, Chandra Kant; Ajith, Parameswaran; Pozzo, Walter Del; Berry, Christopher P L; Nielsen, Alex B; London, Lionel (2018-01-11). "Testing general relativity using gravitational wave signals from the inspiral, merger and ringdown of binary black holes". Classical and Quantum Gravity. 35 (1): 014002. doi:10.1088/1361-6382/aa972e. ISSN 0264-9381.
- Mirshekari, Saeed; Yunes, Nicolás; Will, Clifford M. (2012-01-25). "Constraining Lorentz-violating, modified dispersion relations with gravitational waves". Physical Review D. 85 (2): 024041. doi:10.1103/PhysRevD.85.024041.
- Vinet, Jean-Yves; The Virgo Collaboration (2006). The VIRGO physics book Vol. II (PDF). p. 19.
- T. Accadia; et al. (2012). "Virgo: a laser interferometer to detect gravitational waves". Journal of Instrumentation. 7 (3): P03012. Bibcode:2012JInst...7.3012A. doi:10.1088/1748-0221/7/03/P03012.
- G. Vajente (2008). Analysis of sensitivity and noise sources for the Virgo gravitational wave interferometer (PDF).
- Accadia, T.; Acernese, F.; Antonucci, F.; et al. (2011). "Performance of the Virgo interferometer longitudinal control system during the second science run". Astroparticle Physics. 34 (7): 521–527. Bibcode:2011APh....34..521A. doi:10.1016/j.astropartphys.2010.11.006. ISSN 0927-6505.
- Patrice Hello (1996). Couplings in interferometric gravitational wave detectors (PDF).
- Robinet, F.; et al. (2010). "Data quality in gravitational wave bursts and inspiral searches in the second Virgo Science Run". Class. Quantum Grav. 27 (19): 194012. Bibcode:2010CQGra..27s4012R. doi:10.1088/0264-9381/27/19/194012. S2CID 120922616.
- F. Bondu; et al. (1996). "Ultrahigh-spectral-purity laser for the VIRGO experiment". Optics Letters. 21 (8): 582–4. Bibcode:1996OptL...21..582B. doi:10.1364/OL.21.000582. PMID 19876090.
- F. Bondu; et al. (2002). "The VIRGO injection system" (PDF). Classical and Quantum Gravity. 19 (7): 1829–1833. Bibcode:2002CQGra..19.1829B. doi:10.1088/0264-9381/19/7/381. S2CID 250902832.
- Wei, Li-Wei (2015-12-03). High-power laser system for Advanced Virgo gravitational wave detector : coherently combined master oscillator fiber power amplifiers (PhD thesis). Université Nice Sophia Antipolis.
- "Optical Layout – Virgo". www.virgo-gw.eu. Retrieved 2023-03-05.
- J. Degallaix (2015). "Silicon, the test mass substrate of tomorrow?" (PDF). The Next Detectors for Gravitational Wave Astronomy. Archived from the original (PDF) on 2015-12-08. Retrieved 2015-12-16.
- R. Bonnand (2012). The Advanced Virgo Gravitational Wave Detector/ Study of the optical design and development of the mirrors (PhD) (in French). Université Claude Bernard – Lyon I.
- R Flaminio; et al. (2010). "A study of coating mechanical and optical losses in view of reducing mirror thermal noise in gravitational wave detectors" (PDF). Classical and Quantum Gravity. 27 (8): 084030. Bibcode:2010CQGra..27h4030F. doi:10.1088/0264-9381/27/8/084030. S2CID 122750664.
- M. Lorenzini & Virgo Collaboration (2010). "The monolithic suspension for the virgo interferometer". Classical and Quantum Gravity. 27 (8): 084021. Bibcode:2010CQGra..27h4021L. doi:10.1088/0264-9381/27/8/084021. S2CID 123269358.
- Braccini, S.; Barsotti, L.; Bradaschia, C.; Cella, G.; Virgilio, A. Di; Ferrante, I.; Fidecaro, F.; Fiori, I.; Frasconi, F.; Gennai, A.; Giazotto, A.; Paoletti, F.; Passaquieti, R.; Passuello, D.; Poggiani, R. (2005-07-01). "Measurement of the seismic attenuation performance of the VIRGO Superattenuator". Astroparticle Physics. 23 (6): 557–565. doi:10.1016/j.astropartphys.2005.04.002. ISSN 0927-6505.
- "Quantum Noise Reduction – Virgo". www.virgo-gw.eu. Retrieved 2023-04-07.
- Zhao, Yuhang; Aritomi, Naoki; Capocasa, Eleonora; Leonardi, Matteo; Eisenmann, Marc; Guo, Yuefan; Polini, Eleonora; Tomura, Akihiro; Arai, Koji; Aso, Yoichi; Huang, Yao-Chin; Lee, Ray-Kuang; Lück, Harald; Miyakawa, Osamu; Prat, Pierre (2020-04-28). "Frequency-Dependent Squeezed Vacuum Source for Broadband Quantum Noise Reduction in Advanced Gravitational-Wave Detectors". Physical Review Letters. 124 (17): 171101. arXiv:2003.10672. Bibcode:2020PhRvL.124q1101Z. doi:10.1103/PhysRevLett.124.171101. PMID 32412296. S2CID 214623227.
- Polini, E (2021-08-01). "Broadband quantum noise reduction via frequency dependent squeezing for Advanced Virgo Plus". Physica Scripta. 96 (8): 084003. Bibcode:2021PhyS...96h4003P. doi:10.1088/1402-4896/abfef0. ISSN 0031-8949. S2CID 235285860.
- Virgo Collaboration; Acernese, F.; Agathos, M.; Aiello, L.; Allocca, A.; Amato, A.; Ansoldi, S.; Antier, S.; Arène, M.; Arnaud, N.; Ascenzi, S.; Astone, P.; Aubin, F.; Babak, S.; Bacon, P. (2019-12-05). "Increasing the Astrophysical Reach of the Advanced Virgo Detector via the Application of Squeezed Vacuum States of Light". Physical Review Letters. 123 (23): 231108. Bibcode:2019PhRvL.123w1108A. doi:10.1103/PhysRevLett.123.231108. PMID 31868444. S2CID 209446443.
- "Fighting Noises – Virgo". www.virgo-gw.eu. Retrieved 2023-02-21.
- Private communication from Carlo Bradaschia, Virgo vacuum group leader (2015).
- VIRGO Vacuum System Overview, A.Pasqualetti https://workarea.ego-gw.it/ego2/virgo/advanced-virgo/vac/varies/Virgo_Vacuum_system_Overview_r2.pdf
- Kelly, Thu-Lan; Veitch, Peter J.; Brooks, Aidan F.; Munch, Jesper (2007-02-20). "Accurate and precise optical testing with a differential Hartmann wavefront sensor". Applied Optics. 46 (6): 861–866. Bibcode:2007ApOpt..46..861K. doi:10.1364/AO.46.000861. hdl:2440/43095. ISSN 2155-3165. PMID 17279130.
- Lawrence, Ryan Christopher (2003). Active wavefront correction in laser interferometric gravitational wave detectors (PhD thesis). Massachusetts Institute of Technology. hdl:1721.1/29308.
- Nardecchia, Ilaria (2022). "Detecting Gravitational Waves with Advanced Virgo". Galaxies. 10 (1): 28. Bibcode:2022Galax..10...28N. doi:10.3390/galaxies10010028. ISSN 2075-4434.
- Vinet, Jean-Yves; Brisson, Violette; Braccini, Stefano; Ferrante, Isidoro; Pinard, Laurent; Bondu, François; Tournié, Eric (1997-11-15). "Scattered light noise in gravitational wave interferometric detectors: A statistical approach". Physical Review D. 56 (10): 6085–6095. Bibcode:1997PhRvD..56.6085V. doi:10.1103/PhysRevD.56.6085.
- Vinet, Jean-Yves; Brisson, Violette; Braccini, Stefano (1996-07-15). "Scattered light noise in gravitational wave interferometric detectors: Coherent effects". Physical Review D. 54 (2): 1276–1286. Bibcode:1996PhRvD..54.1276V. doi:10.1103/PhysRevD.54.1276. PMID 10020804.
- Acernese, F.; Amico, P.; Alshourbagy, M.; Antonucci, F.; Aoudia, S.; Astone, P.; Avino, S.; Babusci, D.; Ballardin, G.; Barone, F.; Barsotti, L.; Barsuglia, M.; Bauer, Th. S.; Beauville, F.; Bigotta, S. (April 2007). "Data Acquisition System of the Virgo Gravitational Waves Interferometric Detector". 2007 15th IEEE-NPSS Real-Time Conference: 1–8. doi:10.1109/RTC.2007.4382842. ISBN 978-1-4244-0866-5. S2CID 140107498.
- "O2 Instrumental Lines". www.gw-openscience.org. Retrieved 2023-03-24.
- "Virgo Logbook - Detector Characterisation (Spectral lines)". logbook.virgo-gw.eu. Retrieved 2023-03-24.
- Davis, D; Littenberg, T B; Romero-Shaw, I M; Millhouse, M; McIver, J; Di Renzo, F; Ashton, G (2022-12-15). "Subtracting glitches from gravitational-wave detector data during the third LIGO-Virgo observing run". Classical and Quantum Gravity. 39 (24): 245013. arXiv:2207.03429. Bibcode:2022CQGra..39x5013D. doi:10.1088/1361-6382/aca238. ISSN 0264-9381. S2CID 250334515.
- "Virgo Sensitivity Curves". 2011. Archived from the original on 1 December 2015. Retrieved 15 December 2015.
- Chen, Hsin-Yu; Holz, Daniel E; Miller, John; Evans, Matthew; Vitale, Salvatore; Creighton, Jolien (2021-03-04). "Distance measures in gravitational-wave astrophysics and cosmology". Classical and Quantum Gravity. 38 (5): 055010. arXiv:1709.08079. Bibcode:2021CQGra..38e5010C. doi:10.1088/1361-6382/abd594. ISSN 0264-9381. S2CID 119057584.
- Hello, Patrice (1997). Détection des ondes gravitationnelles - Ecole Joliot Curie (PDF) (Report). Retrieved 2023-04-20.
- "Our Collaborations". LIGO Lab | Caltech. Retrieved 2023-02-26.
- "LIGO-M1000066-v27: LIGO Data Management Plan". dcc.ligo.org. Retrieved 2023-02-26.
- "GWOSC". www.gw-openscience.org. Retrieved 2023-03-05.
- The LIGO Scientific Collaboration; the Virgo Collaboration; the KAGRA Collaboration; Abbott, R.; Abe, H.; Acernese, F.; Ackley, K.; Adhicary, S.; Adhikari, N.; Adhikari, R. X.; Adkins, V. K.; Adya, V. B.; Affeldt, C.; Agarwal, D.; Agathos, M. (2023-02-07). "Open data from the third observing run of LIGO, Virgo, KAGRA and GEO". arXiv:2302.03676 [gr-qc].
- The LIGO Scientific Collaboration; the Virgo Collaboration; the KAGRA Collaboration; Abbott, R.; Abbott, T. D.; Acernese, F.; Ackley, K.; Adams, C.; Adhikari, N.; Adhikari, R. X.; Adya, V. B.; Affeldt, C.; Agarwal, D.; Agathos, M.; Agatsuma, K. (2021-11-17). "GWTC-3: Compact Binary Coalescences Observed by LIGO and Virgo During the Second Part of the Third Observing Run". arXiv:2111.03606 [gr-qc].
- Bohé, Alejandro; Shao, Lijing; Taracchini, Andrea; Buonanno, Alessandra; Babak, Stanislav; Harry, Ian W.; Hinder, Ian; Ossokine, Serguei; Pürrer, Michael; Raymond, Vivien; Chu, Tony; Fong, Heather; Kumar, Prayush; Pfeiffer, Harald P.; Boyle, Michael (2017-02-17). "Improved effective-one-body model of spinning, nonprecessing binary black holes for the era of gravitational-wave astrophysics with advanced detectors". Physical Review D. 95 (4): 044028. arXiv:1611.03703. Bibcode:2017PhRvD..95d4028B. doi:10.1103/PhysRevD.95.044028. S2CID 30505492.
- Husa, Sascha; Khan, Sebastian; Hannam, Mark; Pürrer, Michael; Ohme, Frank; Forteza, Xisco Jiménez; Bohé, Alejandro (2016-02-03). "Frequency-domain gravitational waves from nonprecessing black-hole binaries. I. New numerical waveforms and anatomy of the signal". Physical Review D. 93 (4): 044006. arXiv:1508.07250. Bibcode:2016PhRvD..93d4006H. doi:10.1103/PhysRevD.93.044006. S2CID 118429997.
- Coogan, Adam; Edwards, Thomas D. P.; Chia, Horng Sheng; George, Richard N.; Freese, Katherine; Messick, Cody; Setzer, Christian N.; Weniger, Christoph; Zimmerman, Aaron (2022-12-01). "Efficient gravitational wave template bank generation with differentiable waveforms". Physical Review D. 106 (12): 122001. arXiv:2202.09380. Bibcode:2022PhRvD.106l2001C. doi:10.1103/PhysRevD.106.122001. S2CID 254096550.
- Klimenko, S; Yakushin, I; Mercer, A; Mitselmakher, G (2008-06-07). "A coherent method for detection of gravitational wave bursts". Classical and Quantum Gravity. 25 (11): 114029. arXiv:0802.3232. Bibcode:2008CQGra..25k4029K. doi:10.1088/0264-9381/25/11/114029. ISSN 0264-9381. S2CID 209833580.
- "GraceDB | The Gravitational-Wave Candidate Event Database". gracedb.ligo.org. Retrieved 2023-02-28.
- "Data Analysis - IGWN | Public Alerts User Guide". emfollow.docs.ligo.org. Retrieved 2023-02-28.
- "GCN - General Coordinates Network". gcn.nasa.gov. Retrieved 2023-02-28.
- "Scalable Cyberinfrastructure for Multi-messenger Astrophysics". Scalable Cyberinfrastructure for Multi-messenger Astrophysics. Retrieved 2023-02-28.
- Abbott, R.; Abbott, T. D.; Acernese, F.; Ackley, K.; Adams, C.; Adhikari, N.; Adhikari, R. X.; Adya, V. B.; Affeldt, C.; Agarwal, D.; Agathos, M.; Agatsuma, K.; Aggarwal, N.; Aguiar, O. D.; Aiello, L. (2022-04-01). "Search for Gravitational Waves Associated with Gamma-Ray Bursts Detected by Fermi and Swift during the LIGO–Virgo Run O3b". The Astrophysical Journal. 928 (2): 186. arXiv:2111.03608. Bibcode:2022ApJ...928..186A. doi:10.3847/1538-4357/ac532b. ISSN 0004-637X. S2CID 243832929.
- Williamson, A. R.; Biwer, C.; Fairhurst, S.; Harry, I. W.; Macdonald, E.; Macleod, D.; Predoi, V. (2014-12-24). "Improved methods for detecting gravitational waves associated with short gamma-ray bursts". Physical Review D. 90 (12): 122004. arXiv:1410.6042. Bibcode:2014PhRvD..90l2004W. doi:10.1103/PhysRevD.90.122004. S2CID 86867428.
- Wąs, Michał; Sutton, Patrick J.; Jones, Gareth; Leonor, Isabel (2012-07-23). "Performance of an externally triggered gravitational-wave burst search". Physical Review D. 86 (2): 022003. arXiv:1201.5599. Bibcode:2012PhRvD..86b2003W. doi:10.1103/PhysRevD.86.022003. S2CID 119158252.
- Cho, Min-A. (2019). Low-Latency Searches for Gravitational Waves and their Electromagnetic Counterparts with Advanced LIGO and Virgo (Thesis). Digital Repository at the University of Maryland. Bibcode:2019PhDT........52C. doi:10.13016/7lp5-glut.
- Countryman, Stefan; Keivani, Azadeh; Bartos, Imre; Marka, Zsuzsa; Kintscher, Thomas; Corley, Rainer; Blaufuss, Erik; Finley, Chad; Marka, Szabolcs (2019-01-16). "Low-Latency Algorithm for Multi-messenger Astrophysics (LLAMA) with Gravitational-Wave and High-Energy Neutrino Candidates". arXiv:1901.05486 [astro-ph.HE].
- "Matched filter and signal-to-noise for a periodic template". Noise to signal. 2016-08-25. Retrieved 2023-03-29.
- Dhurandhar, Sanjeev; Krishnan, Badri; Mukhopadhyay, Himan; Whelan, John T. (2008-04-17). "Cross-correlation search for periodic gravitational waves". Physical Review D. 77 (8): 082001. arXiv:0712.1578. Bibcode:2008PhRvD..77h2001D. doi:10.1103/PhysRevD.77.082001. hdl:11858/00-001M-0000-0013-626B-F. S2CID 41261478.
- Romano, Joseph D.; Cornish, Neil. J. (2017). "Detection methods for stochastic gravitational-wave backgrounds: a unified treatment". Living Reviews in Relativity. 20 (1): 2. arXiv:1608.06889. Bibcode:2017LRR....20....2R. doi:10.1007/s41114-017-0004-1. ISSN 2367-3613. PMC 5478100. PMID 28690422.
- Renzini, Arianna I.; Goncharov, Boris; Jenkins, Alexander C.; Meyers, Patrick M. (2022). "Stochastic Gravitational-Wave Backgrounds: Current Detection Efforts and Future Prospects". Galaxies. 10 (1): 34. arXiv:2202.00178. Bibcode:2022Galax..10...34R. doi:10.3390/galaxies10010034. ISSN 2075-4434.
- Gray, Rachel; Hernandez, Ignacio Magaña; Qi, Hong; Sur, Ankan; Brady, Patrick R.; Chen, Hsin-Yu; Farr, Will M.; Fishbach, Maya; Gair, Jonathan R.; Ghosh, Archisman; Holz, Daniel E.; Mastrogiovanni, Simone; Messenger, Christopher; Steer, Danièle A.; Veitch, John (2020-06-08). "Cosmological inference using gravitational wave standard sirens: A mock data analysis". Physical Review D. 101 (12): 122001. doi:10.1103/PhysRevD.101.122001.
- Gray, Rachel; Messenger, Chris; Veitch, John (2022-03-21). "A Pixelated Approach to Galaxy Catalogue Incompleteness: Improving the Dark Siren Measurement of the Hubble Constant". Monthly Notices of the Royal Astronomical Society. 512 (1): 1127–1140. doi:10.1093/mnras/stac366. ISSN 0035-8711.
- Mastrogiovanni, S.; Leyde, K.; Karathanasis, C.; Chassande-Mottin, E.; Steer, D. A.; Gair, J.; Ghosh, A.; Gray, R.; Mukherjee, S.; Rinaldi, S. (2021-09-20). "On the importance of source population models for gravitational-wave cosmology". Physical Review D. 104 (6): 062009. doi:10.1103/PhysRevD.104.062009.
- "Astronomers Catch Gravitational Waves from Colliding Neutron Stars". Sky & Telescope. 2017-10-16. Retrieved 2023-02-20.
- Watson, Darach; Hansen, Camilla J.; Selsing, Jonatan; Koch, Andreas; Malesani, Daniele B.; Andersen, Anja C.; Fynbo, Johan P. U.; Arcones, Almudena; Bauswein, Andreas; Covino, Stefano; Grado, Aniello; Heintz, Kasper E.; Hunt, Leslie; Kouveliotou, Chryssa; Leloudas, Giorgos (October 2019). "Identification of strontium in the merger of two neutron stars". Nature. 574 (7779): 497–500. arXiv:1910.10510. Bibcode:2019Natur.574..497W. doi:10.1038/s41586-019-1676-3. ISSN 1476-4687. PMID 31645733. S2CID 204837882.
- Abbott, B. P.; Abbott, R.; Abbott, T. D.; Acernese, F.; Ackley, K.; Adams, C.; Adams, T.; Addesso, P.; Adhikari, R. X.; Adya, V. B.; Affeldt, C.; Afrough, M.; Agarwal, B.; Agathos, M.; Agatsuma, K. (2017-10-16). "Gravitational Waves and Gamma-Rays from a Binary Neutron Star Merger: GW170817 and GRB 170817A". The Astrophysical Journal. 848 (2): L13. arXiv:1710.05834. Bibcode:2017ApJ...848L..13A. doi:10.3847/2041-8213/aa920c. ISSN 2041-8213. S2CID 126310483.
- LIGO Scientific Collaboration, Virgo Collaboration, and KAGRA Collaboration; Abbott, R.; Abe, H.; Acernese, F.; Ackley, K.; Adhikari, N.; Adhikari, R. X.; Adkins, V. K.; Adya, V. B.; Affeldt, C.; Agarwal, D.; Agathos, M.; Agatsuma, K.; Aggarwal, N.; Aguiar, O. D. (2022-11-28). "All-sky search for continuous gravitational waves from isolated neutron stars using Advanced LIGO and Advanced Virgo O3 data". Physical Review D. 106 (10): 102008. arXiv:2201.00697. Bibcode:2022PhRvD.106j2008A. doi:10.1103/PhysRevD.106.102008. S2CID 245650351.
- Whelan, John T.; Sundaresan, Santosh; Zhang, Yuanhao; Peiris, Prabath (2015-05-20). "Model-based cross-correlation search for gravitational waves from Scorpius X-1". Physical Review D. 91 (10): 102005. arXiv:1504.05890. Bibcode:2015PhRvD..91j2005W. doi:10.1103/PhysRevD.91.102005. S2CID 59360101.
- Abbott, R.; Abe, H.; Acernese, F.; Ackley, K.; Adhikari, N.; Adhikari, R. X.; Adkins, V. K.; Adya, V. B.; Affeldt, C.; Agarwal, D.; Agathos, M.; Agatsuma, K.; Aggarwal, N.; Aguiar, O. D.; Aiello, L. (2022-05-25). "Searches for Gravitational Waves from Known Pulsars at Two Harmonics in the Second and Third LIGO-Virgo Observing Runs". The Astrophysical Journal. 935 (1): 1. arXiv:2111.13106. Bibcode:2022ApJ...935....1A. doi:10.3847/1538-4357/ac6acf. ISSN 0004-637X. S2CID 244709285.
- "LIGO Scientific Collaboration - The science of LSC research". www.ligo.org. Retrieved 2023-03-29.
- LIGO Scientific Collaboration and Virgo Collaboration; Abbott, R.; Abbott, T. D.; Acernese, F.; Ackley, K.; Adams, C.; Adhikari, N.; Adhikari, R. X.; Adya, V. B.; Affeldt, C.; Agarwal, D.; Agathos, M.; Agatsuma, K.; Aggarwal, N.; Aguiar, O. D. (2022-04-28). "Search of the early O3 LIGO data for continuous gravitational waves from the Cassiopeia A and Vela Jr. supernova remnants". Physical Review D. 105 (8): 082005. arXiv:2111.15116. Bibcode:2022PhRvD.105h2005A. doi:10.1103/PhysRevD.105.082005. S2CID 244729269.
- LIGO Scientific Collaboration, Virgo Collaboration, and KAGRA Collaboration; Abbott, R.; Abe, H.; Acernese, F.; Ackley, K.; Adhikari, N.; Adhikari, R. X.; Adkins, V. K.; Adya, V. B.; Affeldt, C.; Agarwal, D.; Agathos, M.; Agatsuma, K.; Aggarwal, N.; Aguiar, O. D. (2022-08-09). "Search for continuous gravitational wave emission from the Milky Way center in O3 LIGO-Virgo data". Physical Review D. 106 (4): 042003. arXiv:2204.04523. Bibcode:2022PhRvD.106d2003A. doi:10.1103/PhysRevD.106.042003. S2CID 248085352.
- Abbott, R.; Abbott, T. D.; Abraham, S.; Acernese, F.; Ackley, K.; Adams, A.; Adams, C.; Adhikari, R. X.; Adya, V. B.; Affeldt, C.; Agarwal, D.; Agathos, M.; Agatsuma, K.; Aggarwal, N.; Aguiar, O. D. (2021-07-23). "Upper limits on the isotropic gravitational-wave background from Advanced LIGO and Advanced Virgo's third observing run". Physical Review D. 104 (2): 022004. arXiv:2101.12130. Bibcode:2021PhRvD.104b2004A. doi:10.1103/PhysRevD.104.022004. ISSN 2470-0010. S2CID 231719405.
- The LIGO Scientific Collaboration; the Virgo Collaboration; the KAGRA Collaboration; Abbott, R.; Abe, H.; Acernese, F.; Ackley, K.; Adhikari, N.; Adhikari, R. X.; Adkins, V. K.; Adya, V. B.; Affeldt, C.; Agarwal, D.; Agathos, M.; Agatsuma, K. (2021-11-19). "Constraints on the cosmic expansion history from GWTC-3". arXiv:2111.03604 [astro-ph, physics:gr-qc]. doi:10.48550/arxiv.2111.03604.
- "Outreach – Virgo". www.virgo-gw.eu. Retrieved 2023-05-08.
- "Festa di Scienza e Filosofia". Festa di Scienza e Filosofia. Retrieved 2023-05-08.
- "Gravitional waves: Windows to the universe". Athens Science Festival. Retrieved 2023-05-08.
- Rossi, Giada (2022-11-23). "Black Hole: a new interactive installation by EGO and INFN at Città della Scienza in Naples". EGO - European Gravitational Observatory. Retrieved 2023-05-08.
- "Home page". Il Ritmo Dello Spazio. Retrieved 2023-02-26.
- "On Air". Studio Tomás Sarceno. 2018-10-13. Retrieved 2023-02-26.
- "Guided Tour". EGO - European Gravitational Observatory. Retrieved 2023-02-26.
- "International Day of Women and Girls in Science 2023 – Virgo". www.virgo-gw.eu. Retrieved 2023-02-26.